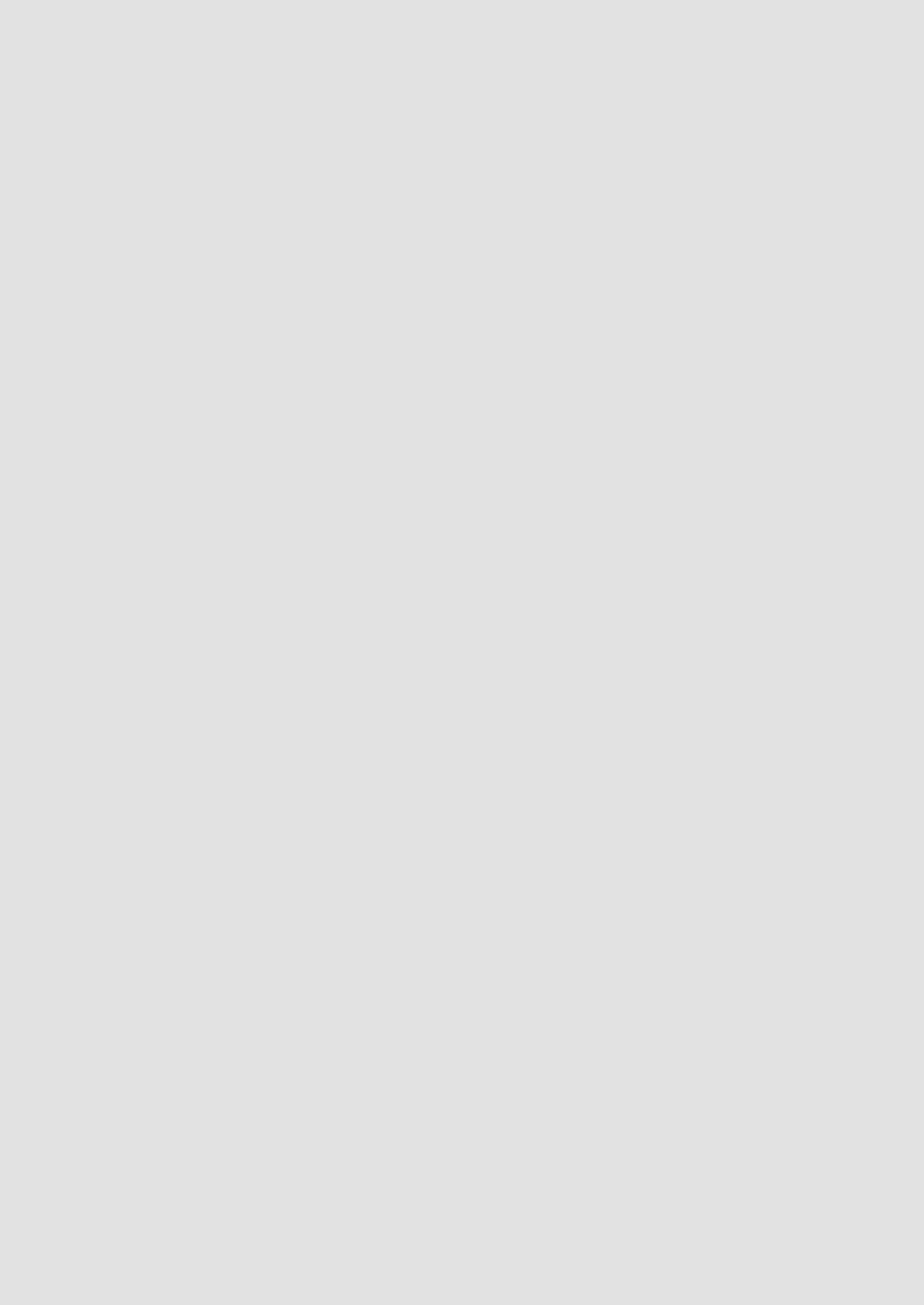
257
REFERENCES
IRENA Coalition for Action (2020a), Companies in transition towards 100% renewable energy: Focus
on heating and cooling, white paper, IRENA, Abu Dhabi.
IRENA Coalition for Action (2020b), Stimulating investment in community energy: Broadening
the ownership of renewables, white paper, IRENA, Abu Dhabi.
IRENA Coalition for Action (2018), Community Energy: Broadening the Ownership of Renewables,
IRENA, Abu Dhabi.
IRENA and CPI (2020), Global Landscape of Renewable Energy Finance 2020, IRENA, Climate
Policy Initiative, Abu Dhabi and London.
IRENA and CPI (2018), Global Landscape of Renewable Energy Finance 2018, IRENA, CPI, Abu Dhabi
and London.
IRENA and IEA-PVPS (2016), End-of-Life Management: Solar Photovoltaic Panels, IRENA,
International Energy Agency - Photovoltaic Power Systems, Abu Dhabi and Paris.
IRENA, IEA and REN21 (2020), Renewable Energy Policies in a Time of Transition: Heating and
Cooling, IRENA, OECD/IEA and Renewable Energy Policy Network for the 21st Century, Abu Dhabi
and Paris.
IRENA, IEA and REN21 (2018), Renewable Energy Policies in a Time of Transition, IRENA, OECD/
IEA and REN21, Abu Dhabi and Paris.
Jolly, J. (2021), “Major UK pension funds worth nearly GBP 900 bn commit to net zero?”, 10 March,
The Guardian, www.theguardian.com/business/2021/mar/10/major-uk-pension-funds-worth-
nearly-900bn-commit-to-net-zero.
Lederman, J. and D. Chow. (2021), “Biden commits to cutting U.S. emissions in half by 2030 as
part of Paris climate pact”, NBC news, 22 April, www.nbcnews.com/politics/white-house/biden-
will-commit-halving-u-s-emissions-2030-part-paris-n1264892.
Li S. and Yu R. (2021), “China reveals co-operation with EU on green investment standards”,
7 April, Financial Times, www.ft.com/content/cddd464f-9a37-41a0-8f35-62d98fa0cca0.
Louie, E. and Pearce, J. (2016), “Retraining investment for U.S. transition from coal to solar
photovoltaic employment”, Energy Economics, Volume 57, Pages 295-302, ISSN 0140-9883,
https://doi.org/10.1016/j.eneco.2016.05.016.
Mao, X. D. Rutherford, L. Osipova, and B. Comer (2020), “Refueling assessment of a zero-
emission container corridor between China and the United States: Could hydrogen replace fossil
fuels?”, International Council for Clean Transportation, Working Paper 2020-05, https://theicct.org/
sites/default/files/publications/Zero-emission-container-corridor-hydrogen-3.5.2020.pdf.
Marston J. (2018), “Why these companies turn to coal country for skilled workers”, www.edf.org/
blog/2018/05/08/why-these-companies-turn-coal-country-skilled-workers.
Mazzacurati, E. (2017), “Art. 173: France’s Groundbreaking Climate Risk Reporting Law”,
16 January, Four Twenty Seven, www.427mt.com/2017/01/16/impact-french-law-article-173/
(accessed 26 June 2021).
McLaughlin, C. (2019), “Denmark ends district heating heat pump grants”, Ammonia21,
10 January, www.ammonia21.com/articles/8776/denmark_ends_district_heating_heat_pump_
grants#:~:text=Denmark%20has%20ended%20its%20support,for%20district%20heating%20
heat%20pumps.
MNRE (2021), “Production linked incentive scheme ‘National Programme on High Efficiency Solar
PV Modules’”, F. No. 283/62/2020-grid solar, Ministry of New & Renewable Energy, Government of
India, https://mnre.gov.in/img/documents/uploads/file_f-1619672166750.pdf.
Müller, F., S. Claar, M. Neumann and C. Elsner (2020), “Is green a Pan-African colour? Mapping
African renewable energy policies and transitions in 34 countries”, Energy Research & Social
Science, Vol. 68, 101551, https://doi.org/10.1016/j.erss.2020.101551.